Role and Evolution of the Extracellular Matrix in the Acquisition of Complex Multicellularity in Eukaryotes: A Macroalgal Perspective
Keywords
Article abstract
Multicellular eukaryotes are characterized by an expanded extracellular matrix (ECM) with a diversified composition. The ECM is involved in determining tissue texture, screening cells from the outside medium, development, and innate immunity, all of which are essential features in the biology of multicellular eukaryotes. This review addresses the origin and evolution of the ECM, with a focus on multicellular marine algae. We show that in these lineages the expansion of extracellular matrix played a major role in the acquisition of complex multicellularity through its capacity to connect, position, shield, and defend the cells. Multiple innovations were necessary during these evolutionary processes, leading to striking convergences in the structures and functions of the ECMs of algae, animals, and plants.
Article content
1. Introduction
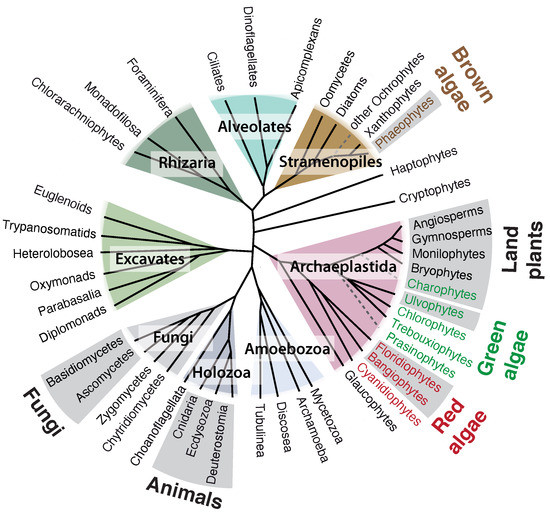
2. Structure of the Extracellular Matrix of Marine Macroalgae
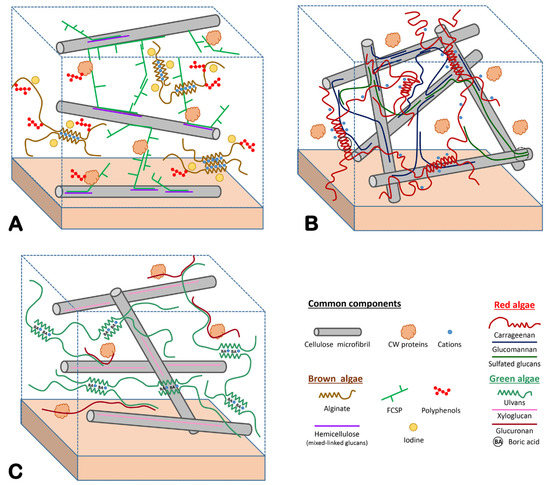
3. Origin of ECM Components in Plants and Algae
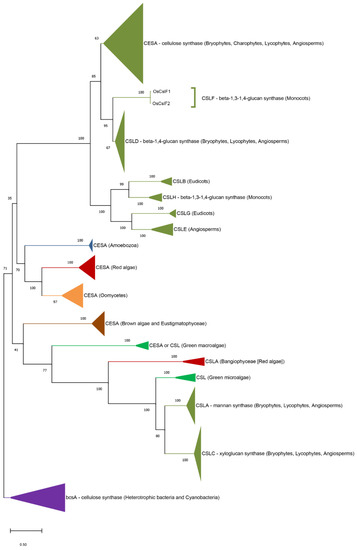
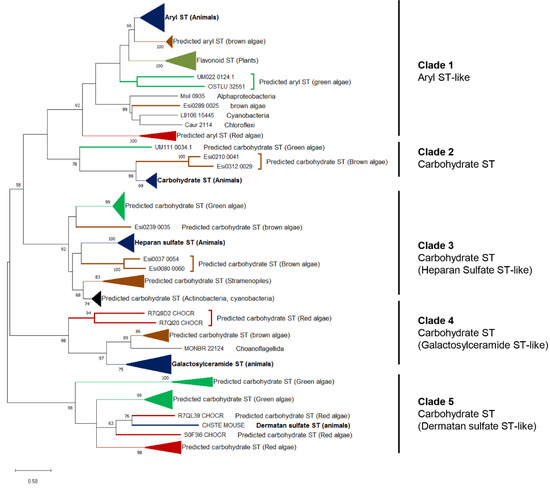
4. Biomechanical Properties of the ECM
5. Interfacing with the External Medium
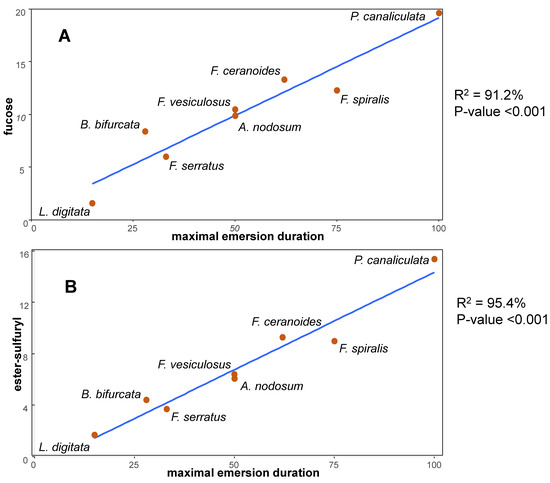
6. ECM Signaling and Development
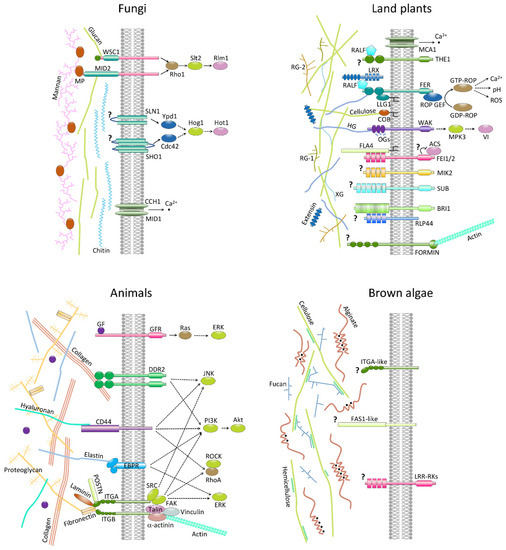
7. ECM Signaling and Innate Immunity
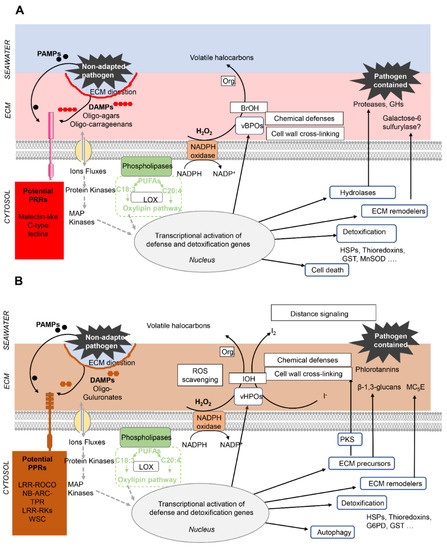
8. Conclusions
Supplementary Materials
Author Contributions
Funding
Institutional Review Board Statement
Informed Consent Statement
Data Availability Statement
Acknowledgments
Conflicts of Interest
References
- Grosberg, R.K.; Strathmann, R.R. The evolution of multicellularity: A minor major transition? Annu. Rev. Ecol. Evol. Syst. 2007, 38, 621–654. [Google Scholar] [CrossRef][Green Version]
- Cock, J.M.; Collen, J. Independent emergence of complex multicellularity in the brown and red algae. In Evolutionary Transitions to Multicellular Life; Ruiz-Trillo, I., Nedelcu, A.M., Eds.; Springer: Dordrecht, The Netherlands, 2015; pp. 335–361. [Google Scholar]
- Coelho, S.M.; Cock, J.M. Brown Algal Model Organisms. Annu. Rev. Genet. 2020, 54, 71–92. [Google Scholar] [CrossRef]
- Michel, G.; Tonon, T.; Scornet, D.; Cock, J.M.; Kloareg, B. The cell wall polysaccharide metabolism of the brown alga Ectocarpus Siliculosus. Insights into the evolution of extracellular matrix polysaccharides in Eukaryotes. New Phytol. 2010, 188, 82–97. [Google Scholar] [CrossRef] [PubMed]
- Cock, J.M.; Sterck, L.; Rouze, P.; Scornet, D.; Allen, A.E.; Amoutzias, G.; Anthouard, V.; Artiguenave, F.; Aury, J.M.; Badger, J.H.; et al. The Ectocarpus genome and the independent evolution of multicellularity in brown algae. Nature 2010, 465, 617–621. [Google Scholar] [CrossRef] [PubMed][Green Version]
- Collen, J.; Porcel, B.; Carre, W.; Ball, S.G.; Chaparro, C.; Tonon, T.; Barbeyron, T.; Michel, G.; Noel, B.; Valentin, K.; et al. Genome structure and metabolic features in the red seaweed Chondrus crispus shed light on evolution of the Archaeplastida. Proc. Natl. Acad. Sci. USA 2013, 110, 5247–5252. [Google Scholar] [CrossRef] [PubMed][Green Version]
- Brawley, S.H.; Blouin, N.A.; Ficko-Blean, E.; Wheeler, G.L.; Lohr, M.; Goodson, H.V.; Jenkins, J.W.; Blaby-Haas, C.E.; Helliwell, K.E.; Chan, C.X.; et al. Insights into the red algae and eukaryotic evolution from the genome of Porphyra umbilicalis (Bangiophyceae, Rhodophyta). Proc. Natl. Acad. Sci. USA 2017, 114, E6361–E6370. [Google Scholar] [CrossRef] [PubMed][Green Version]
- De Clerck, O.; Kao, S.M.; Bogaert, K.A.; Blomme, J.; Foflonker, F.; Kwantes, M.; Vancaester, E.; Vanderstraeten, L.; Aydogdu, E.; Boesger, J.; et al. Insights into the Evolution of Multicellularity from the Sea Lettuce Genome. Curr. Biol. 2018, 28, 2921–2933. [Google Scholar] [CrossRef][Green Version]
- Nishiyama, T.; Sakayama, H.; de Vries, J.; Buschmann, H.; Saint-Marcoux, D.; Ullrich, K.K.; Haas, F.B.; Vanderstraeten, L.; Becker, D.; Lang, D.; et al. The Chara Genome: Secondary Complexity and Implications for Plant Terrestrialization. Cell 2018, 174, 448–464. [Google Scholar] [CrossRef][Green Version]
- Karamanos, N.K.; Theocharis, A.D.; Piperigkou, Z.; Manou, D.; Passi, A.; Skandalis, S.S.; Vynios, D.H.; Orian-Rousseau, V.; Ricard-Blum, S.; Schmelzer, C.E.H.; et al. A guide to the composition and functions of the extracellular matrix. FEBS J. 2021. [Google Scholar] [CrossRef]
- Carpita, N.C.; McCann, M. The cell wall. In Biochemistry & Molecular Biology of Plants; Buchanan, B., Gruissem, W., Jones, R., Eds.; American Society of Plant Physiologists: Rockville, MD, USA, 2000; pp. 52–108. [Google Scholar]
- Popper, Z.A.; Michel, G.; Herve, C.; Domozych, D.S.; Willats, W.G.; Tuohy, M.G.; Kloareg, B.; Stengel, D.B. Evolution and diversity of plant cell walls: From algae to flowering plants. Annu. Rev. Plant Biol. 2011, 62, 567–590. [Google Scholar] [CrossRef][Green Version]
- Gow, N.A.R.; Latge, J.P.; Munro, C.A. The Fungal Cell Wall: Structure, Biosynthesis, and Function. Microbiolology Spectr. 2017, 5. [Google Scholar] [CrossRef][Green Version]
- Cronshaw, J.; Myers, A.; Preston, R.D. A chemical and physical investigation of the cell walls of some marine algae. Biochim. Biophys. Acta 1958, 27, 89–103. [Google Scholar] [CrossRef]
- Kloareg, B.; Quatrano, R.S. Structure of the cell walls of marine algae and ecophysiological functions of the matrix polysaccharides. Oceanogr. Mar. Biol. Ann. Rev. 1988, 26, 259–315. [Google Scholar]
- Ficko-Blean, E.; Hervé, C.; Michel, G. Sweet and sour sugars from the sea: The biosynthesis and remodeling of sulfated cell wall polysaccharides from marine macroalgae. Perspect. Phycol. 2015, 2, 51–64. [Google Scholar] [CrossRef]
- Haug, A.; Larsen, B.; Smidsrød, O. Uronic acid sequence in alginate from different sources. Carbohydr. Res. 1974, 32, 217–225. [Google Scholar] [CrossRef]
- Deniaud-Bouet, E.; Hardouin, K.; Potin, P.; Kloareg, B.; Herve, C. A review about brown algal cell walls and fucose-containing sulfated polysaccharides: Cell wall context, biomedical properties and key research challenges. Carbohydr. Polym. 2017, 175, 395–408. [Google Scholar] [CrossRef]
- Chevolot, L.; Mulloy, B.; Ratiskol, J.; Foucault, A.; Colliec-Jouault, S. A disaccharide repeat unit is the major structure in fucoidans from two species of brown algae. Carbohydr. Res. 2001, 330, 529–535. [Google Scholar] [CrossRef]
- Bilan, M.I.; Grachev, A.A.; Ustuzhanina, N.E.; Shashkov, A.S.; Nifantiev, N.E.; Usov, A.I. Structure of a fucoidan from the brown seaweed Fucus evanescens C.Ag. Carbohydr. Res. 2002, 337, 719–730. [Google Scholar] [CrossRef]
- Colin, S.; Deniaud, E.; Jam, M.; Descamps, V.; Chevolot, Y.; Kervarec, N.; Yvin, J.C.; Barbeyron, T.; Michel, G.; Kloareg, B. Cloning and biochemical characterization of the fucanase FcnA: Definition of a novel glycoside hydrolase family specific for sulfated fucans. Glycobiology 2006, 16, 1021–1032. [Google Scholar] [CrossRef]
- Nishino, T.; Nagumo, T.; Kiyohara, H.; Yamada, H. Structural characterization of a new anticoagulant fucan sulfate from the brown seaweed Ecklonia Kurome. Carbohydr. Res. 1991, 211, 77–90. [Google Scholar] [CrossRef]
- Anastyuk, S.D.; Shevchenko, N.M.; Nazarenko, E.L.; Imbs, T.I.; Gorbach, V.I.; Dmitrenok, P.S.; Zvyagintseva, T.N. Structural analysis of a highly sulfated fucan from the brown alga Laminaria cichorioides by tandem MALDI and ESI mass spectrometry. Carbohydr. Res. 2010, 345, 2206–2212. [Google Scholar] [CrossRef]
- Nagaoka, M.; Shibata, H.; Kimura-Takagi, I.; Hashimoto, S.; Kimura, K.; Makino, T.; Aiyama, R.; Ueyama, S.; Yokokura, T. Structural study of fucoidan from Cladosiphon okamuranus TOKIDA. Glycoconj. J. 1999, 16, 19–26. [Google Scholar] [CrossRef] [PubMed]
- Sakai, T.; Ishizuka, K.; Shimanaka, K.; Ikai, K.; Kato, I. Structures of oligosaccharides derived from Cladosiphon okamuranus fucoidan by digestion with marine bacterial enzymes. Mar. Biotechnol. 2003, 5, 536–544. [Google Scholar]
- Deniaud-Bouet, E.; Kervarec, N.; Michel, G.; Tonon, T.; Kloareg, B.; Herve, C. Chemical and enzymatic fractionation of cell walls from Fucales: Insights into the structure of the extracellular matrix of brown algae. Ann. Bot. 2014, 114, 1203–1216. [Google Scholar] [CrossRef] [PubMed][Green Version]
- Raimundo, S.C.; Pattathil, S.; Eberhard, S.; Hahn, M.G.; Popper, Z.A. β-1,3-Glucans are components of brown seaweed (Phaeophyceae) cell walls. Protoplasma 2017, 254, 997–1016. [Google Scholar] [CrossRef] [PubMed]
- Salmean, A.A.; Duffieux, D.; Harholt, J.; Qin, F.; Michel, G.; Czjzek, M.; Willats, W.G.T.; Herve, C. Insoluble (1 --> 3), (1 --> 4)-β-D-glucan is a component of cell walls in brown algae (Phaeophyceae) and is masked by alginates in tissues. Sci. Rep. 2017, 7, 2880. [Google Scholar] [CrossRef] [PubMed]
- Herve, C.; Simeon, A.; Jam, M.; Cassin, A.; Johnson, K.L.; Salmean, A.A.; Willats, W.G.; Doblin, M.S.; Bacic, A.; Kloareg, B. Arabinogalactan proteins have deep roots in eukaryotes: Identification of genes and epitopes in brown algae and their role in Fucus serratus embryo development. New Phytol. 2016, 209, 1428–1441. [Google Scholar] [CrossRef] [PubMed]
- Ford, L.; Theodoridou, K.; Sheldrake, G.N.; Walsh, P.J. A critical review of analytical methods used for the chemical characterisation and quantification of phlorotannin compounds in brown seaweeds. Phytochem. Anal. 2019, 30, 587–599. [Google Scholar] [CrossRef]
- Verhaeghe, E.F.; Fraysse, A.; Guerquin-Kern, J.L.; Wu, T.D.; Deves, G.; Mioskowski, C.; Leblanc, C.; Ortega, R.; Ambroise, Y.; Potin, P. Microchemical imaging of iodine distribution in the brown alga Laminaria digitata suggests a new mechanism for its accumulation. J. Biol. Inorg. Chem. 2008, 13, 257–269. [Google Scholar] [CrossRef] [PubMed]
- Lechat, H. Nature Et Organisation Des Polysaccharides Parietaux De L’algue Rouge Eucheuma Cottonii. Ph.D. Thesis, Université de Nantes, Nantes, France, 1998. [Google Scholar]
- Lechat, H.; Amat, M.; Mazoyer, J.; Buléon, A.; Lahaye, M. Structure and distribution of glucomannan and sulfated glucan in the cell walls of the red alga Kappaphycus alvarezii (Gigartinales, Rhodophyta). J. Phycol. 2000, 36, 891–902. [Google Scholar] [CrossRef]
- Diener, M.; Adamcik, J.; Sanchez-Ferrer, A.; Jaedig, F.; Schefer, L.; Mezzenga, R. Primary, Secondary, Tertiary and Quaternary Structure Levels in Linear Polysaccharides: From Random Coil, to Single Helix to Supramolecular Assembly. Biomacromolecules 2019, 20, 1731–1739. [Google Scholar] [CrossRef] [PubMed]
- Lahaye, M.; Robic, A. Structure and functional properties of ulvan, a polysaccharide from green seaweeds. Biomacromolecules 2007, 8, 1765–1774. [Google Scholar] [CrossRef] [PubMed]
- Craigie, J. Cell Walls. In Biology of the Red Algae; Cole, K., Sheath, R., Eds.; Cambridge University Press: Cambridge, UK, 1990; pp. 221–257. [Google Scholar]
- Rees, D. Structure, conformation, and mechanism in the formation of polysaccharide gels and networks. Adv. Carbohydr. Chem. Biochem. 1969, 24, 267–332. [Google Scholar] [PubMed]
- Lahaye, M.; Yaphe, W.; Viet, M.T.P.; Rochas, C. C-13-N-M-R Spectroscopic Investigation of Methylated and Charged Agarose Oligosaccharides and Polysaccharides. Carbohydr. Res. 1989, 190, 249–265. [Google Scholar] [CrossRef]
- Van de Velde, F.; Knutsen, S.; Usov, A.; Rollema, H.; Cerezo, A. 1H and 13C high resolution NMR spectroscopy of carrageenans: Application in research and industry. Trends Food Sci. Tech. 2002, 13, 73–92. [Google Scholar] [CrossRef]
- Anderson, N.S.; Rees, D.A. Porphyran—A Polysaccharide with a Masked Repeating Structure. J. Chem. Soc. 1965, 5880–5887. [Google Scholar] [CrossRef]
- Knutsen, S.; Myslabodski, D.; Larsen, B.; Usov, A. A modified system of nomenclature for red algal galactans. Bot. Mar. 1994, 37, 163–169. [Google Scholar] [CrossRef]
- Michel, G.; Nyval-Collen, P.; Barbeyron, T.; Czjzek, M.; Helbert, W. Bioconversion of red seaweed galactans: A focus on bacterial agarases and carrageenases. Appl. Microbiol. Biotechnol. 2006, 71, 23–33. [Google Scholar] [CrossRef] [PubMed]
- Hehemann, J.H.; Correc, G.; Barbeyron, T.; Helbert, W.; Czjzek, M.; Michel, G. Transfer of carbohydrate-active enzymes from marine bacteria to Japanese gut microbiota. Nature 2010, 464, 908–912. [Google Scholar] [CrossRef]
- Ficko-Blean, E.; Prechoux, A.; Thomas, F.; Rochat, T.; Larocque, R.; Zhu, Y.; Stam, M.; Genicot, S.; Jam, M.; Calteau, A.; et al. Carrageenan catabolism is encoded by a complex regulon in marine heterotrophic bacteria. Nat. Commun. 2017, 8, 1685. [Google Scholar] [CrossRef]
- Ropartz, D.; Giuliani, A.; Herve, C.; Geairon, A.; Jam, M.; Czjzek, M.; Rogniaux, H. High-energy photon activation tandem mass spectrometry provides unprecedented insights into the structure of highly sulfated oligosaccharides extracted from macroalgal cell walls. Anal. Chem. 2015, 87, 1042–1049. [Google Scholar] [CrossRef]
- Ropartz, D.; Giuliani, A.; Fanuel, M.; Herve, C.; Czjzek, M.; Rogniaux, H. Online coupling of high-resolution chromatography with extreme UV photon activation tandem mass spectrometry: Application to the structural investigation of complex glycans by dissociative photoionization. Anal. Chim. Acta 2016, 933, 1–9. [Google Scholar] [CrossRef] [PubMed]
- Naretto, A.; Fanuel, M.; Ropartz, D.; Rogniaux, H.; Larocque, R.; Czjzek, M.; Tellier, C.; Michel, G. The agar-specific hydrolase ZgAgaC from the marine bacterium Zobellia galactanivorans defines a new GH16 protein subfamily. J. Biol. Chem. 2019, 294, 6923–6939. [Google Scholar] [CrossRef] [PubMed][Green Version]
- Correc, G.; Hehemann, J.H.; Czjzek, M.; Helbert, W. Structural analysis of the degradation products of porphyran digested by Zobellia galactanivorans—Porphyranase A. Carbohydr. Polym. 2011, 83, 227–283. [Google Scholar] [CrossRef]
- Guibet, M.; Boulenguer, P.; Mazoyer, J.; Kervarec, N.; Antonopoulos, A.; Lafosse, M.; Helbert, W. Composition and distribution of carrabiose moieties in hybrid kappa-/iota-carrageenans using carrageenases. Biomacromolecules 2008, 9, 408–415. [Google Scholar] [CrossRef]
- Vilen, E.M.; Lundqvist, L.C.; Jouanneau, D.; Helbert, W.; Sandstrom, C. NMR study on hydroxy protons of kappa-and kappa/mu-hybrid carrageenan oligosaccharides: Experimental evidence of hydrogen bonding and chemical exchange interactions in kappa/mu oligosaccharides. Biomacromolecules 2010, 11, 3487–3494. [Google Scholar] [CrossRef] [PubMed]
- Kolender, A.A.; Pujol, C.A.; Damonte, E.B.; Matulewicz, M.C.; Cerezo, A.S. The system of sulfated α-(1-->3)-linked D-mannans from the red seaweed Nothogenia fastigiata: Structures, antiherpetic and anticoagulant properties. Carbohydr. Res. 1997, 304, 53–60. [Google Scholar] [CrossRef]
- Ray, B.; Lahaye, M. Cell-wall polysaccharides from the marine green alga Ulva “rigida” (ulvales, chlorophyta). Extraction and chemical composition. Carbohydr. Res. 1995, 274, 251–261. [Google Scholar] [CrossRef]
- Lahaye, M.; Ray, B.; Inizan, F.; Brunel, M.; Bobin-Dubigeon, C.; Quéméner, B. Chemical structure, localization and associations of “sea-lettuce” (Ulva sp.) dietary fibres. In Profibre: Functional Properties of Non-Digestible Carbohydrates; Guillon, F., Amado, R., Amaral-Collacüo, M.T., Andersson, H., Asp, N.G., Bach Knudsen, K.E., Champ, M., Mathers, J., Robertson, J.A., Rowland, I., et al., Eds.; INRA: Nantes, France, 1998. [Google Scholar]
- Brading, J.W.E.; Georg-Plant, M.M.T.; Hardy, D.M. The polysaccharide from the alga Ulva lactuca. Purification, hydrolysis, and methylation of the polysaccharide. J. Chem. Soc. 1954, 319–324. [Google Scholar] [CrossRef]
- McKinnell, J.P.; Percival, E. Structural investigations on the water-soluble polysaccharide of the green seaweed Enteromorpha compressa. J. Chem. Soc. 1962, 3141–3148. [Google Scholar] [CrossRef]
- Quemener, B.; Lahaye, M.; Bobin-Dubigeon, C. Sugar determination in ulvans by a chemical-enzymatic method coupled to high performance anion exchange chromatography. J. Appl. Phycol. 1997, 9, 179–188. [Google Scholar] [CrossRef]
- Nyvall Collen, P.; Sassi, J.F.; Rogniaux, H.; Marfaing, H.; Helbert, W. Ulvan lyases isolated from the Flavobacteria Persicivirga ulvanivorans are the first members of a new polysaccharide lyase family. J. Biol. Chem. 2011, 286, 42063–42071. [Google Scholar] [CrossRef] [PubMed][Green Version]
- Rydahl, M.G.; Krac Un, S.K.; Fangel, J.U.; Michel, G.; Guillouzo, A.; Genicot, S.; Mravec, J.; Harholt, J.; Wilkens, C.; Motawia, M.S.; et al. Development of novel monoclonal antibodies against starch and ulvan—implications for antibody production against polysaccharides with limited immunogenicity. Sci. Rep. 2017, 7, 9326. [Google Scholar] [CrossRef] [PubMed][Green Version]
- Ulaganathan, T.; Boniecki, M.T.; Foran, E.; Buravenkov, V.; Mizrachi, N.; Banin, E.; Helbert, W.; Cygler, M. New Ulvan-Degrading Polysaccharide Lyase Family: Structure and Catalytic Mechanism Suggests Convergent Evolution of Active Site Architecture. ACS Chem. Biol. 2017, 12, 1269–1280. [Google Scholar] [CrossRef]
- Ulaganathan, T.; Banin, E.; Helbert, W.; Cygler, M. Structural and functional characterization of PL28 family ulvan lyase NLR48 from Nonlabens ulvanivorans. J. Biol. Chem. 2018, 293, 11564–11573. [Google Scholar] [CrossRef] [PubMed][Green Version]
- Ulaganathan, T.; Helbert, W.; Kopel, M.; Banin, E.; Cygler, M. Structure-function analyses of a PL24 family ulvan lyase reveal key features and suggest its catalytic mechanism. J. Biol. Chem. 2018, 293, 4026–4036. [Google Scholar] [CrossRef] [PubMed][Green Version]
- Reisky, L.; Prechoux, A.; Zuhlke, M.K.; Baumgen, M.; Robb, C.S.; Gerlach, N.; Roret, T.; Stanetty, C.; Larocque, R.; Michel, G.; et al. A marine bacterial enzymatic cascade degrades the algal polysaccharide ulvan. Nat. Chem. Biol. 2019, 15, 803–812. [Google Scholar] [CrossRef] [PubMed][Green Version]
- Tenhaken, R.; Voglas, E.; Cock, J.M.; Neu, V.; Huber, C.G. Characterization of GDP-mannose dehydrogenase from the brown alga Ectocarpus siliculosus providing the precursor for the alginate polymer. J. Biol. Chem. 2011, 286, 16707–16715. [Google Scholar] [CrossRef][Green Version]
- Nyvall, P.; Corre, E.; Boisset, C.; Barbeyron, T.; Rousvoal, S.; Scornet, D.; Kloareg, B.; Boyen, C. Characterization of mannuronan C-5-epimerase genes from the brown alga Laminaria digitata. Plant Physiol. 2003, 133, 726–735. [Google Scholar] [CrossRef] [PubMed][Green Version]
- Fischl, R.; Bertelsen, K.; Gaillard, F.; Coelho, S.; Michel, G.; Klinger, M.; Boyen, C.; Czjzek, M.; Herve, C. The cell-wall active mannuronan C5-epimerases in the model brown alga Ectocarpus: From gene context to recombinant protein. Glycobiology 2016, 26, 973–983. [Google Scholar] [CrossRef] [PubMed][Green Version]
- Meslet-Cladiere, L.; Delage, L.; Leroux, C.J.; Goulitquer, S.; Leblanc, C.; Creis, E.; Gall, E.A.; Stiger-Pouvreau, V.; Czjzek, M.; Potin, P. Structure/function analysis of a type iii polyketide synthase in the brown alga Ectocarpus siliculosus reveals a biochemical pathway in phlorotannin monomer biosynthesis. Plant Cell 2013, 25, 3089–30103. [Google Scholar] [CrossRef] [PubMed][Green Version]
- Rees, D.A. Enzymic synthesis of 3:6-anhydro-l-galactose within porphyran from l-galactose 6-sulphate units. Biochem. J. 1961, 81, 347–352. [Google Scholar] [CrossRef] [PubMed][Green Version]
- Lawson, C.J.; Rees, D.A. An enzyme for the metabolic control of polysaccharide conformation and function. Nature 1970, 227, 392–393. [Google Scholar] [CrossRef] [PubMed]
- Genicot-Joncour, S.; Poinas, A.; Richard, O.; Potin, P.; Rudolph, B.; Kloareg, B.; Helbert, W. The cyclization of the 3,6-anhydro-galactose ring of iota-carrageenan is catalyzed by two D-galactose-2,6-sulfurylases in the red alga Chondrus crispus. Plant Physiol. 2009, 151, 1609–1616. [Google Scholar] [CrossRef][Green Version]
- Lombard, V.; Golaconda Ramulu, H.; Drula, E.; Coutinho, P.M.; Henrissat, B. The carbohydrate-active enzymes database (CAZy) in 2013. Nucleic Acids Res. 2014, 42, D490–D495. [Google Scholar] [CrossRef][Green Version]
- CAZypedia_Consortium. Ten years of CAZypedia: A living encyclopedia of carbohydrate-active enzymes. Glycobiology 2018, 28, 3–8. [Google Scholar] [CrossRef]
- Dhugga, K.S.; Barreiro, R.; Whitten, B.; Stecca, K.; Hazebroek, J.; Randhawa, G.S.; Dolan, M.; Kinney, A.J.; Tomes, D.; Nichols, S.; et al. Guar seed β-mannan synthase is a member of the cellulose synthase super gene family. Science 2004, 303, 363–366. [Google Scholar] [CrossRef]
- Cocuron, J.C.; Lerouxel, O.; Drakakaki, G.; Alonso, A.P.; Liepman, A.H.; Keegstra, K.; Raikhel, N.; Wilkerson, C.G. A gene from the cellulose synthase-like C family encodes a β-1,4 glucan synthase. Proc. Natl. Acad. Sci. USA 2007, 104, 8550–8555. [Google Scholar] [CrossRef] [PubMed][Green Version]
- Yang, J.; Bak, G.; Burgin, T.; Barnes, W.J.; Mayes, H.B.; Pena, M.J.; Urbanowicz, B.R.; Nielsen, E. Biochemical and Genetic Analysis Identify CSLD3 as a β-1,4-Glucan Synthase That Functions during Plant Cell Wall Synthesis. Plant Cell 2020, 32, 1749–1767. [Google Scholar] [CrossRef] [PubMed][Green Version]
- Burton, R.A.; Wilson, S.M.; Hrmova, M.; Harvey, A.J.; Shirley, N.J.; Medhurst, A.; Stone, B.A.; Newbigin, E.J.; Bacic, A.; Fincher, G.B. Cellulose synthase-like CslF genes mediate the synthesis of cell wall (1,3;1,4)-β-D-glucans. Science 2006, 311, 1940–1942. [Google Scholar] [CrossRef] [PubMed]
- Doblin, M.S.; Pettolino, F.A.; Wilson, S.M.; Campbell, R.; Burton, R.A.; Fincher, G.B.; Newbigin, E.; Bacic, A. A barley cellulose synthase-like CSLH gene mediates (1,3;1,4)-β-D-glucan synthesis in transgenic Arabidopsis. Proc. Natl. Acad. Sci. USA 2009, 106, 5996–6001. [Google Scholar] [CrossRef] [PubMed][Green Version]
- Roberts, E.; Roberts, A.W. A cellulose synthase (CESA) gene from the red alga Porphyra yezoensis (Rhodophyta). J.Phycol. 2009, 45, 203–212. [Google Scholar] [CrossRef] [PubMed]
- Matthews, P.R.; Schindler, M.; Howles, P.; Arioli, T.; Williamson, R.E. A CESA from Griffithsia monilis (Rhodophyta, Florideophyceae) has a family 48 carbohydrate-binding module. J. Exp. Bot. 2010, 61, 4461–4468. [Google Scholar] [CrossRef] [PubMed][Green Version]
- Nobles, D.R.; Romanovicz, D.K.; Brown, R.M., Jr. Cellulose in cyanobacteria. Origin of vascular plant cellulose synthase? Plant Physiol. 2001, 127, 529–542. [Google Scholar] [CrossRef] [PubMed]
- Nobles, D.R.; Brown, R.M. The pivotal role of cyanobacteria in the evolution of cellulose synthases and cellulose synthase-like proteins. Cellulose 2004, 11, 437–448. [Google Scholar] [CrossRef]
- Yin, Y.; Huang, J.; Xu, Y. The cellulose synthase superfamily in fully sequenced plants and algae. BMC Plant Biol. 2009, 9, 99–112. [Google Scholar] [CrossRef] [PubMed][Green Version]
- Kumar, S.; Stecher, G.; Li, M.; Knyaz, C.; Tamura, K. MEGA X: Molecular Evolutionary Genetics Analysis across Computing Platforms. Mol. Biol. Evol. 2018, 35, 1547–1549. [Google Scholar] [CrossRef] [PubMed]
- Popper, Z.A.; Tuohy, M.G. Beyond the green: Understanding the evolutionary puzzle of plant and algal cell walls. Plant Physiol. 2010, 153, 373–383. [Google Scholar] [CrossRef][Green Version]
- Habuchi, O. Diversity and functions of glycosaminoglycan sulfotransferases. Biochim. Biophys. Acta 2000, 1474, 115–127. [Google Scholar] [CrossRef]
- Liu, J.; Moon, A.F.; Sheng, J.; Pedersen, L.C. Understanding the substrate specificity of the heparan sulfate sulfotransferases by an integrated biosynthetic and crystallographic approach. Curr. Opin. Struct. Biol. 2012, 22, 550–557. [Google Scholar] [CrossRef][Green Version]
- Olsen, J.L.; Rouze, P.; Verhelst, B.; Lin, Y.C.; Bayer, T.; Collen, J.; Dattolo, E.; De Paoli, E.; Dittami, S.; Maumus, F.; et al. The genome of the seagrass Zostera marina reveals angiosperm adaptation to the sea. Nature 2016, 530, 331–335. [Google Scholar] [CrossRef] [PubMed][Green Version]
- Michel, G.; Tonon, T.; Scornet, D.; Cock, J.M.; Kloareg, B. Central and storage carbon metabolism of the brown alga Ectocarpus siliculosus: Insights into the origin and evolution of storage carbohydrates in Eukaryotes. New Phytol. 2010, 188, 67–81. [Google Scholar] [CrossRef] [PubMed]
- Charrier, B.; Le Bail, A.; de Reviers, B. Plant Proteus: Brown algal morphological plasticity and underlying developmental mechanisms. Trends Plant Sci. 2012, 17, 468–477. [Google Scholar] [CrossRef] [PubMed]
- Rees, D.A. Shapely polysaccharides. The eighth Colworth medal lecture. Biochem. J. 1972, 126, 257–273. [Google Scholar] [CrossRef] [PubMed][Green Version]
- Ertesvag, H. Alginate-modifying enzymes: Biological roles and biotechnological uses. Front. Microbiol. 2015, 6, 523. [Google Scholar]
- Haug, A.; Larsen, B. Biosynthesis of alginate. Epimerisation of D-mannuronic to L-guluronic acid residues in the polymer chain. Biochim. Biophys. Acta 1969, 192, 557–559. [Google Scholar] [CrossRef]
- Ye, N.; Zhang, X.; Miao, M.; Fan, X.; Zheng, Y.; Xu, D.; Wang, J.; Zhou, L.; Wang, D.; Gao, Y.; et al. Saccharina genomes provide novel insight into kelp biology. Nat. Commun. 2015, 6, 6986. [Google Scholar] [CrossRef] [PubMed][Green Version]
- Shao, Z.; Zhang, P.; Lu, C.; Li, S.; Chen, Z.; Wang, X.; Duan, D. Transcriptome sequencing of Saccharina japonica sporophytes during whole developmental periods reveals regulatory networks underlying alginate and mannitol biosynthesis. BMC Genom. 2019, 20, 975. [Google Scholar] [CrossRef][Green Version]
- Tonon, T.; Rousvoal, S.; Roeder, V.; Boyen, C. Expression Profiling of the Mannuronan C5-Epimerase Multigenic Family in the Brown Alga Laminaria Digitata (Phaeophyceae) under Biotic Stress Conditions. J. Phycol. 2008, 44, 1250–1256. [Google Scholar] [CrossRef]
- Cosse, A.; Potin, P.; Leblanc, C. Patterns of gene expression induced by oligoguluronates reveal conserved and environment-specific molecular defense responses in the brown alga Laminaria digitata. New Phytol. 2009, 182, 239–250. [Google Scholar] [CrossRef]
- Rees, D.A. Enzymic desulphation of porphyran. Biochem. J. 1961, 80, 449–453. [Google Scholar] [CrossRef] [PubMed]
- Lipinska, A.P.; Collen, J.; Krueger-Hadfield, S.A.; Mora, T.; Ficko-Blean, E. To gel or not to gel: Differential expression of carrageenan-related genes between the gametophyte and tetasporophyte life cycle stages of the red alga Chondrus crispus. Sci. Rep. 2020, 10, 11498. [Google Scholar] [CrossRef] [PubMed]
- Canty, E.G.; Kadler, K.E. Procollagen trafficking, processing and fibrillogenesis. J. Cell Sci. 2005, 118, 1341–1353. [Google Scholar] [CrossRef][Green Version]
- Broder, C.; Arnold, P.; Vadon-Le Goff, S.; Konerding, M.A.; Bahr, K.; Muller, S.; Overall, C.M.; Bond, J.S.; Koudelka, T.; Tholey, A.; et al. Metalloproteases meprin α and meprin β are C- and N-procollagen proteinases important for collagen assembly and tensile strength. Proc. Natl. Acad. Sci. USA 2013, 110, 14219–14224. [Google Scholar] [CrossRef] [PubMed][Green Version]
- Wolf, S.; Mouille, G.; Pelloux, J. Homogalacturonan methyl-esterification and plant development. Mol. Plant. 2009, 2, 851–860. [Google Scholar] [CrossRef] [PubMed]
- Muller, K.; Levesque-Tremblay, G.; Bartels, S.; Weitbrecht, K.; Wormit, A.; Usadel, B.; Haughn, G.; Kermode, A.R. Demethylesterification of cell wall pectins in Arabidopsis plays a role in seed germination. Plant Physiol. 2013, 161, 305–316. [Google Scholar] [CrossRef] [PubMed][Green Version]
- Yokoyama, R. A Genomic Perspective on the Evolutionary Diversity of the Plant Cell Wall. Plants 2020, 9, 1195. [Google Scholar] [CrossRef] [PubMed]
- Domozych, D.S.; Sun, L.; Palacio-Lopez, K.; Reed, R.; Jeon, S.; Li, M.; Jiao, C.; Sorensen, I.; Fei, Z.; Rose, J.K.C. Endomembrane architecture and dynamics during secretion of the extracellular matrix of the unicellular charophyte, Penium Margaritaceum. J. Exp. Bot. 2020, 71, 3323–3339. [Google Scholar] [CrossRef] [PubMed]
- McCarthy, T.W.; Der, J.P.; Honaas, L.A.; de Pamphilis, C.W.; Anderson, C.T. Phylogenetic analysis of pectin-related gene families in Physcomitrella patens and nine other plant species yields evolutionary insights into cell walls. BMC Plant Biol. 2014, 14, 79. [Google Scholar] [CrossRef][Green Version]
- Chahine, N.O.; Chen, F.H.; Hung, C.T.; Ateshian, G.A. Direct measurement of osmotic pressure of glycosaminoglycan solutions by membrane osmometry at room temperature. Biophys. J. 2005, 89, 1543–1550. [Google Scholar] [CrossRef][Green Version]
- Pomin, V.H. Keratan sulfate: An up-to-date review. Int. J. Biol. Macromol. 2015, 72, 282–289. [Google Scholar] [CrossRef] [PubMed]
- Maytin, E.V. Hyaluronan: More than just a wrinkle filler. Glycobiology 2016, 26, 553–559. [Google Scholar] [CrossRef] [PubMed][Green Version]
- Kloareg, B. Isolation and analysis of cell walls of the brown marine algae Pelvetia canaliculata and Ascophyllum nodosum. Physiol. Végétale 1984, 22, 47–56. [Google Scholar]
- Mabeau, S.; Kloareg, B. Isolation and Analysis of the Cell Walls of Brown Algae: Fucus spiralis, F. ceranoides, F. vesiculosus, F. serratus, Bifurcaria bifurcata and Laminaria digitata. J. Exp. Bot. 1987, 38, 1573–1580. [Google Scholar] [CrossRef]
- Kloareg, B.; Demarty, M.; Mabeau, S. Ion-Exchange Properties of Isolated Cell Walls of Brown Algae: The Interstitial Solution. J. Exp. Bot. 1987, 38, 1652–1662. [Google Scholar] [CrossRef]
- Kowacz, M.; Pollack, G.H. Cells in New Light: Ion Concentration, Voltage, and Pressure Gradients across a Hydrogel Membrane. ACS Omega 2020, 5, 21024–21031. [Google Scholar] [CrossRef]
- Dittami, S.M.; Gravot, A.; Goulitquer, S.; Rousvoal, S.; Peters, A.F.; Bouchereau, A.; Boyen, C.; Tonon, T. Towards deciphering dynamic changes and evolutionary mechanisms involved in the adaptation to low salinities in Ectocarpus (brown algae). Plant J. 2012, 71, 366–377. [Google Scholar] [CrossRef]
- Torode, T.A.; Marcus, S.E.; Jam, M.; Tonon, T.; Blackburn, R.S.; Herve, C.; Knox, J.P. Monoclonal antibodies directed to fucoidan preparations from brown algae. PLoS ONE 2015, 10, e0118366. [Google Scholar] [CrossRef][Green Version]
- Simeon, A.; Kridi, S.; Kloareg, B.; Herve, C. Presence of Exogenous Sulfate Is Mandatory for Tip Growth in the Brown Alga Ectocarpus subulatus. Front. Plant Sci. 2020, 11, 1277. [Google Scholar] [CrossRef] [PubMed]
- Aquino, R.S.; Landeira-Fernandez, A.M.; Valente, A.P.; Andrade, L.R.; Mourao, P.A. Occurrence of sulfated galactans in marine angiosperms: Evolutionary implications. Glycobiology 2005, 15, 11–20. [Google Scholar] [CrossRef] [PubMed][Green Version]
- Aquino, R.S.; Grativol, C.; Mourao, P.A. Rising from the sea: Correlations between sulfated polysaccharides and salinity in plants. PLoS ONE 2011, 6, e18862. [Google Scholar] [CrossRef] [PubMed][Green Version]
- Hamann, T. The plant cell wall integrity maintenance mechanism—A case study of a cell wall plasma membrane signaling network. Phytochemistry 2015, 112, 100–109. [Google Scholar] [CrossRef] [PubMed]
- Rui, Y.; Dinneny, J.R. A wall with integrity: Surveillance and maintenance of the plant cell wall under stress. New Phytol. 2020, 225, 1428–1439. [Google Scholar] [CrossRef] [PubMed][Green Version]
- Hastings, J.F.; Skhinas, J.N.; Fey, D.; Croucher, D.R.; Cox, T.R. The extracellular matrix as a key regulator of intracellular signalling networks. Br. J. Pharmacol. 2019, 176, 82–92. [Google Scholar] [CrossRef] [PubMed][Green Version]
- Kleiser, S.; Nystrom, A. Interplay between Cell-Surface Receptors and Extracellular Matrix in Skin. Biomolecules 2020, 10, 1170. [Google Scholar] [CrossRef] [PubMed]
- Kropf, D.L.; Kloareg, B.; Quatrano, R.S. Cell wall is required for fixation of the embryonic axis in Fucus zygotes. Science 1988, 239, 187–190. [Google Scholar] [CrossRef]
- Berger, F.; Taylor, A.; Brownlee, C. Cell Fate Determination by the Cell Wall in Early Fucus Development. Science 1994, 263, 1421–1423. [Google Scholar] [CrossRef] [PubMed]
- Bouget, F.Y.; Berger, F.; Brownlee, C. Position dependent control of cell fate in the Fucus embryo: Role of intercellular communication. Development 1998, 125, 1999–2008. [Google Scholar] [CrossRef]
- Arun, A.; Peters, N.T.; Scornet, D.; Peters, A.F.; Mark Cock, J.; Coelho, S.M. Non-cell autonomous regulation of life cycle transitions in the model brown alga Ectocarpus. New Phytol. 2013, 197, 503–510. [Google Scholar] [CrossRef] [PubMed][Green Version]
- Yao, H.; Scornet, D.; Jam, M.; Herve, C.; Potin, P.; Oliveira Correia, L.; Coelho, S.M.; Cock, J.M. Biochemical characteristics of a diffusible factor that induces gametophyte to sporophyte switching in the brown alga Ectocarpus. J. Phycol. 2021, in press. [Google Scholar] [CrossRef]
- Jones, J.D.; Vance, R.E.; Dangl, J.L. Intracellular innate immune surveillance devices in plants and animals. Science 2016, 354. [Google Scholar] [CrossRef] [PubMed][Green Version]
- Ausubel, F.M. Are innate immune signaling pathways in plants and animals conserved? Nat. Immunol. 2005, 6, 973–979. [Google Scholar] [CrossRef]
- Tomlin, H.; Piccinini, A.M. A complex interplay between the extracellular matrix and the innate immune response to microbial pathogens. Immunology 2018, 155, 186–201. [Google Scholar] [CrossRef] [PubMed]
- Nurnberger, T.; Brunner, F.; Kemmerling, B.; Piater, L. Innate immunity in plants and animals: Striking similarities and obvious differences. Immunol. Rev. 2004, 198, 249–266. [Google Scholar] [CrossRef] [PubMed]
- Dangl, J.L.; Horvath, D.M.; Staskawicz, B.J. Pivoting the plant immune system from dissection to deployment. Science 2013, 341, 746–751. [Google Scholar] [CrossRef][Green Version]
- Jones, J.D.; Dangl, J.L. The plant immune system. Nature 2006, 444, 323–329. [Google Scholar] [CrossRef][Green Version]
- Boller, T.; He, S.Y. Innate immunity in plants: An arms race between pattern recognition receptors in plants and effectors in microbial pathogens. Science 2009, 324, 742–744. [Google Scholar] [CrossRef][Green Version]
- Gachon, C.M.; Sime-Ngando, T.; Strittmatter, M.; Chambouvet, A.; Kim, G.H. Algal diseases: Spotlight on a black box. Trends Plant Sci. 2010, 15, 633–640. [Google Scholar] [CrossRef] [PubMed]
- Potin, P.; Bouarab, K.; Salaün, J.-P.; Pohnert, G.; Kloareg, B. Biotic interactions of marine algae. Curr. Opin. Plant Biol. 2002, 5, 308–317. [Google Scholar] [CrossRef]
- Weinberger, F. Pathogen-induced defense and innate immunity in macroalgae. Biol. Bull. 2007, 213, 290–302. [Google Scholar] [CrossRef] [PubMed]
- Cosse, A.; Leblanc, C.; Potin, P. Dynamic defense of marine macroalgae against pathogens: From early activated to gene-regulated responses. Adv. Bot. Res. 2007, 46, 221–266. [Google Scholar]
- Küpper, F.C.; Kloareg, B.; Guern, J.; Potin, P. Oligoguluronates elicit an oxidative burst in the brown algal kelp Laminaria digitata. Plant Physiol. 2001, 125, 278–291. [Google Scholar] [CrossRef][Green Version]
- Küpper, F.C.; Müller, D.G.; Peters, A.F.; Kloareg, B.; Potin, P. Oligoalginate recognition and oxidative burst play a key role in natural and induced resistance of sporophytes of laminariales. J. Chem. Ecol. 2002, 28, 2057–2081. [Google Scholar] [CrossRef]
- Thomas, F.; Cosse, A.; Le Panse, S.; Kloareg, B.; Potin, P.; Leblanc, C. Kelps feature systemic defense responses: Insights into the evolution of innate immunity in multicellular eukaryotes. New Phytol. 2014, 204, 567–576. [Google Scholar] [CrossRef]
- Thomas, F.; Cosse, A.; Goulitquer, S.; Raimund, S.; Morin, P.; Valero, M.; Leblanc, C.; Potin, P. Waterborne signaling primes the expression of elicitor-induced genes and buffers the oxidative responses in the brown alga Laminaria digitata. PLoS ONE 2011, 6, e21475. [Google Scholar] [CrossRef]
- Weinberger, F.; Friedlander, M.; Hoppe, H.-G. Oligoagars elicit a physiological response in gracilaria conferta (rhodophyta). J. Phycol. 1999, 35, 747–755. [Google Scholar] [CrossRef]
- Weinberger, F.; Friedlander, M. Response of Gracilaria conferta (Rhodophyta) to oligoagars results in defense against agar-degrading epiphytes. J. Phycol. 2000, 36, 1079–1086. [Google Scholar] [CrossRef]
- Weinberger, F.; Richard, C.; Kloareg, B.; Kashman, Y.; Hoppe, H.-G.; Friedlander, M. Structure-activity relationships of oligoagar elicitors toward Gracilaria conferta (Rhodophyta). J. Phycol. 2001, 37, 418–426. [Google Scholar] [CrossRef]
- Bouarab, K.; Potin, P.; Correa, J.; Kloareg, B. Sulfated Oligosaccharides Mediate the Interaction between a Marine Red Alga and Its Green Algal Pathogenic Endophyte. Plant Cell 1999, 11, 1635–1650. [Google Scholar] [CrossRef] [PubMed][Green Version]
- Bouarab, K.; Adas, F.; Gaquerel, E.; Kloareg, B.; Salaun, J.P.; Potin, P. The innate immunity of a marine red alga involves oxylipins from both the eicosanoid and octadecanoid pathways. Plant Physiol. 2004, 135, 1838–1848. [Google Scholar] [CrossRef] [PubMed][Green Version]
- Uppalapati, S.R.; Fujita, Y. Carbohydrate regulation of attachment, encystment, and appressorium formation by Pythium porphyrae (Oomycota) zoospores on Porphyra yezoensis (Rhodophyta). J. Phycol. 2000, 36, 359–366. [Google Scholar] [CrossRef]
- Gachon, C.M.; Strittmatter, M.; Muller, D.G.; Kleinteich, J.; Kupper, F.C. Detection of differential host susceptibility to the marine oomycete pathogen Eurychasma dicksonii by real-time PCR: Not all algae are equal. Appl. Environ. Microbiol. 2009, 75, 322–328. [Google Scholar] [CrossRef] [PubMed][Green Version]
- Grenville-Briggs, L.; Gachon, C.M.; Strittmatter, M.; Sterck, L.; Kupper, F.C.; van West, P. A molecular insight into algal-oomycete warfare: cDNA analysis of Ectocarpus siliculosus infected with the basal oomycete Eurychasma dicksonii. PLoS ONE 2011, 6, e24500. [Google Scholar] [CrossRef] [PubMed][Green Version]
- Murua, P.; Muller, D.G.; Etemadi, M.; van West, P.; Gachon, C.M.M. Host and pathogen autophagy are central to the inducible local defences and systemic response of the giant kelp Macrocystis pyrifera against the oomycete pathogen Anisolpidium ectocarpii. New Phytol. 2020, 226, 1445–1460. [Google Scholar] [CrossRef] [PubMed][Green Version]
- Tsirigoti, A.; Beakes, G.W.; Herve, C.; Gachon, C.M.; Katsaros, C. Attachment, penetration and early host defense mechanisms during the infection of filamentous brown algae by Eurychasma dicksonii. Protoplasma 2015, 252, 845–856. [Google Scholar] [CrossRef] [PubMed]
- de Oliveira, L.S.; Tschoeke, D.A.; Magalhaes Lopes, A.C.R.; Sudatti, D.B.; Meirelles, P.M.; Thompson, C.C.; Pereira, R.C.; Thompson, F.L. Molecular Mechanisms for Microbe Recognition and Defense by the Red Seaweed Laurencia dendroidea. Msphere 2017, 2. [Google Scholar] [CrossRef][Green Version]
- Im, S.H.; Klochkova, T.A.; Lee, D.J.; Gachon, C.M.M.; Kim, G.H. Genetic toolkits of the red alga Pyropia tenera against the three most common diseases in Pyropia farms. J. Phycol. 2019, 55, 801–815. [Google Scholar] [CrossRef]
- Tang, L.; Qiu, L.; Liu, C.; Du, G.; Mo, Z.; Tang, X.; Mao, Y. Transcriptomic Insights into Innate Immunity Responding to Red Rot Disease in Red Alga Pyropia Yezoensis. Int. J. Mol. Sci. 2019, 20, 5970. [Google Scholar] [CrossRef] [PubMed][Green Version]
- Leblanc, C.; Colin, C.; Cosse, A.; Delage, L.; La Barre, S.; Morin, P.; Fievet, B.; Voiseux, C.; Ambroise, Y.; Verhaeghe, E.; et al. Iodine transfers in the coastal marine environment: The key role of brown algae and of their vanadium-dependent haloperoxidases. Biochimie 2006, 88, 1773–1785. [Google Scholar] [CrossRef]
- Küpper, F.C.; Schweigert, N.; Ar Gall, E.; Legendre, J.-M.; Vilter, H.; Kloareg, B. Iodine uptake in Laminariales involves extracellular, haloperoxidase-mediated oxidation of iodide. Planta 1998, 207, 163–171. [Google Scholar] [CrossRef]
- Kupper, F.C.; Carpenter, L.J.; McFiggans, G.B.; Palmer, C.J.; Waite, T.J.; Boneberg, E.M.; Woitsch, S.; Weiller, M.; Abela, R.; Grolimund, D.; et al. Iodide accumulation provides kelp with an inorganic antioxidant impacting atmospheric chemistry. Proc. Natl. Acad. Sci. USA 2008, 105, 6954–6958. [Google Scholar] [CrossRef] [PubMed][Green Version]
- Colin, C.; Leblanc, C.; Wagner, E.; Delage, L.; Leize-Wagner, E.; Van Dorsselaer, A.; Kloareg, B.; Potin, P. The brown algal kelp Laminaria digitata features distinct bromoperoxidase and iodoperoxidase activities. J. Biol. Chem. 2003, 278, 23545–23552. [Google Scholar] [CrossRef][Green Version]
- Colin, C.; Leblanc, C.; Michel, G.; Wagner, E.; Leize-Wagner, E.; Van Dorsselaer, A.; Potin, P. Vanadium-dependent iodoperoxidases in Laminaria digitata, a novel biochemical function diverging from brown algal bromoperoxidases. J. Biol. Inorg. Chem. 2005, 10, 156–166. [Google Scholar] [CrossRef] [PubMed]
- Liu, T.; Wang, X.; Wang, G.; Jia, S.; Liu, G.; Shan, G.; Chi, S.; Zhang, J.; Yu, Y.; Xue, T.; et al. Evolution of Complex Thallus Alga: Genome Sequencing of Saccharina japonica. Front. Genet. 2019, 10, 378. [Google Scholar] [CrossRef] [PubMed]
- Salgado, L.T.; Cinelli, L.P.; Viana, N.B.; Tomazetto de Carvalho, R.; De Souza Mourao, P.A.; Teixeira, V.L.; Farina, M.; Filho, A.G. A Vanadium Bromoperoxidase Catalyzes the Formation of High-Molecular-Weight Complexes between Brown Algal Phenolic Substances and Alginates. J. Phycol. 2009, 45, 193–202. [Google Scholar] [CrossRef] [PubMed]
- Zambounis, A.; Elias, M.; Sterck, L.; Maumus, F.; Gachon, C.M. Highly dynamic exon shuffling in candidate pathogen receptors... what if brown algae were capable of adaptive immunity? Mol. Biol. Evol. 2012, 29, 1263–1276. [Google Scholar] [CrossRef]
- Maekawa, T.; Kufer, T.A.; Schulze-Lefert, P. NLR functions in plant and animal immune systems: So far and yet so close. Nat. Immunol. 2011, 12, 817–826. [Google Scholar] [CrossRef] [PubMed]
- Urbach, J.M.; Ausubel, F.M. The NBS-LRR architectures of plant R-proteins and metazoan NLRs evolved in independent events. Proc. Natl. Acad. Sci. USA 2017, 114, 1063–1068. [Google Scholar] [CrossRef][Green Version]
- Herve, C.; Tonon, T.; Collen, J.; Corre, E.; Boyen, C. NADPH oxidases in Eukaryotes: Red algae provide new hints! Curr. Genet. 2006, 49, 190–204. [Google Scholar] [CrossRef]
- Teng, L.; Han, W.; Fan, X.; Xu, D.; Zhang, X.; Dittami, S.M.; Ye, N. Evolution and Expansion of the Prokaryote-Like Lipoxygenase Family in the Brown Alga Saccharina Japonica. Front. Plant Sci. 2017, 8, 2018. [Google Scholar] [CrossRef][Green Version]
- Macaisne, N.; Liu, F.; Scornet, D.; Peters, A.F.; Lipinska, A.; Perrineau, M.M.; Henry, A.; Strittmatter, M.; Coelho, S.M.; Cock, J.M. The Ectocarpus IMMEDIATE UPRIGHT gene encodes a member of a novel family of cysteine-rich proteins with an unusual distribution across the eukaryotes. Development 2017, 144, 409–418. [Google Scholar] [PubMed][Green Version]
- Blomme, J.; Liu, X.; Jacobs, T.B.; De Clerck, O. A molecular toolkit for the green seaweed Ulva mutabilis. Plant. Physiol. 2021, in press. [Google Scholar] [CrossRef] [PubMed]
- Badis, Y.; Scornet, D.; Harada, M.; Caillard, C.; Godfroy, O.; Raphalen, M.; Gachon, C.M.M.; Coelho, S.M.; Motomura, T.; Nagasato, C.; et al. Targeted CRISPR-Cas9-based gene knockouts in the model brown alga Ectocarpus. New Phytol. 2021, in press. [Google Scholar] [CrossRef] [PubMed]